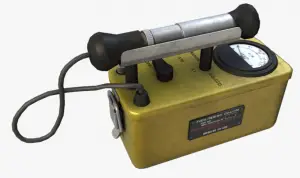
A Geiger counter consists of a Geiger-Müller tube (the sensing element which detects the radiation) and the processing electronics, which displays the result.
Geiger counter can detect ionizing radiation such as alpha and beta particles, neutrons, and gamma rays using the ionization effect produced in a Geiger–Müller tube, which gives its name to the instrument. The voltage of the detector is adjusted so that the conditions correspond to the Geiger-Mueller region.
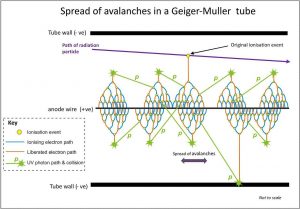
In this region, the voltage is high enough to provide the primary electrons with sufficient acceleration and energy to ionize additional atoms of the medium. These secondary ions (gas amplification) formed are also accelerated, causing an effect known as Townsend avalanches. These avalanches can be triggered and propagated by photons emitted by atoms excited in the original avalanche. Since these photons are not affected by the electric field, they may interact far (e.g., laterally to the axis) from the primary avalanche, and the entire Geiger tube participates in the process.
A strong signal (the amplification factor can reach about 1010) is produced by these avalanches with shape and height independently of the primary ionization and the energy of the detected photon. The voltage pulse, in this case, would be a large and easily detectable ≈ 1.6 V. The technical advantage of a Geiger counter is its simplicity of construction and its insensitivity to small voltage fluctuations. It is very useful for the general measurement of nuclear radiation, but it has two important disadvantages.
-
This diagram shows the number of ion pairs generated in the gas-filled detector, which varies according to the applied voltage for constant incident radiation. The voltages vary widely depending on the detector geometry, gas type, and pressure. This figure schematically indicates the different voltage regions for alpha, beta, and gamma rays. There are six main practical operating regions, where three (ionization, proportional, and Geiger-Mueller region) are useful for detecting ionizing radiation. Alpha particles are more ionizing than beta particles, and gamma rays, so more current is produced in the ion chamber region by alpha than beta and gamma, but the particles cannot be differentiated. More current is produced in the proportional counting region by alpha particles than beta. Still, by the nature of proportional counting, it is possible to differentiate alpha, beta, and gamma pulses. In the Geiger region, there is no differentiation of alpha and beta as any single ionization event in the gas results in the same current output. Since the pulse height is independent of the type and energy of radiation, discrimination is not possible. There is no information on the nature of the ionization that caused the pulse.
- Because of the large avalanche induced by any ionization, a Geiger counter takes a long time (about 1 ms) to recover between successive pulses. Therefore, Geiger counters cannot measure high radiation rates due to the “dead time” of the tube.
There is a subtle but important difference between ionization chambers and Geiger counters. An ionization chamber will produce a current proportional to the number of electrons collected each second (no amplification occurs). This current is averaged and is used to drive a display reading in Bq, or μSv/h. Proportional and Geiger counters do not work in this way. Instead, they amplify each of the individual bursts of ionization so that each ionizing event is detected separately. They, therefore, measure the number of ionizing events (which is why they are called counters). While ionization chambers can be operated in current or pulse mode, proportional counters or Geiger counters are almost always used in pulse mode. In contrast to proportional counters, the G-M counters are mainly used for portable instrumentation due to their sensitivity, simple counting circuit, and ability to detect low-level radiation.
Geiger-Mueller Region
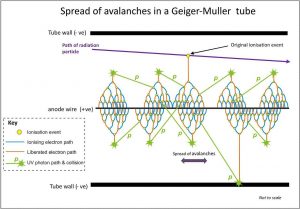
In the Geiger-Mueller region, the voltage and thus the electric field are so strong that secondary avalanches can occur. These avalanches can be triggered and propagated by photons emitted by atoms excited in the original avalanche. Since these photons are not affected by the electric field, they may interact far (e.g., laterally to the axis) from the primary avalanche, and the entire Geiger tube participates in the process. A strong signal (the amplification factor can reach about 1010) is produced by these avalanches with shape and height independently of the primary ionization and the energy of the detected photon. Detectors operated in the Geiger-Mueller region can detect gamma rays and all types of charged particles that can enter the detector. These detectors are known as Geiger counters. The main advantage of these instruments is that they usually do not require any signal amplifiers. Since the positive ions do not move far from the avalanche region, a positively charged ion cloud disturbs the electric field and terminates the avalanche process. The avalanche’s termination is improved by using “quenching” techniques. In contrast to proportional counters, the energy of incident radiation particles can not be distinguished by Geiger counters since the output signal is independent of the amount and type of original ionization.
Basic Principle of Geiger Counters
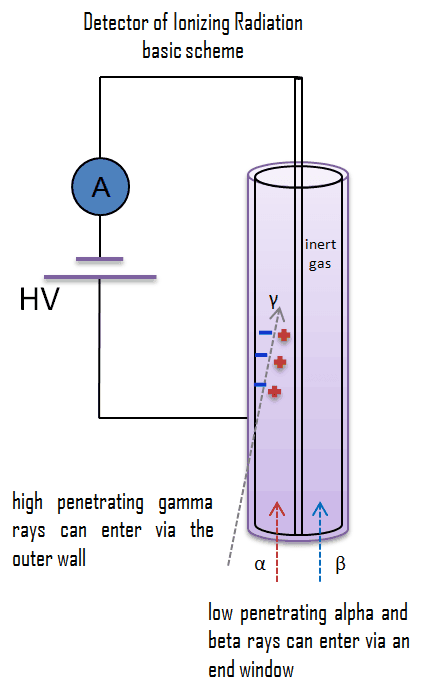
The Geiger counter has a cathode and an anode held at high voltage, and the device is characterized by a capacitance determined by the electrodes’ geometry. In a Geiger counter, the fill gas of the chamber is an inert gas that is ionized by incident radiation and a quench gas of 5–10% of an organic vapor or a halogen gas to prevent spurious pulsing by quenching the electron avalanches.
As ionizing radiation enters the gas between the electrodes, a finite number of ion pairs are formed. The average energy needed to produce an ion in the air is about 34 eV. Therefore a 1 MeV radiation completely absorbed in the detector produces about 3 x 104 pairs of ions. The behavior of the resultant ion pairs is affected by the potential gradient of the electric field within the gas and the type and pressure of the fill gas. Under the influence of the electric field, the positive ions will move toward the negatively charged electrode (outer cylinder), and the negative ions (electrons) will migrate toward the positive electrode (central wire). The electric field in this region keeps the ions from recombining with the electrons. Near the anode wire, the field strength becomes large enough to produce Townsend avalanches. These avalanches can be triggered and propagated by photons emitted by atoms excited in the original avalanche. Since these photons are not affected by the electric field, they may interact far (e.g., laterally to the axis) from the primary avalanche, and the entire Geiger tube participates in the process. A strong signal (the amplification factor can reach about 1010) is produced by these avalanches with shape and height independently of the primary ionization and the energy of the detected photon. The high amplification factor of the Geiger counter is the major advantage over the ionization chamber. The Geiger counter is a much more sensitive device than other chambers. It is often used to detect low-level gamma rays and beta particles for this reason.
Since the positive ions do not move far from the avalanche region, a positively charged ion cloud disturbs the electric field and terminates the avalanche process. The avalanche’s termination is improved by using “quenching” techniques.
Collecting all these electrons will produce a charge on the electrodes and an electrical pulse across the detection circuit. Each pulse corresponds to one gamma-ray or neutron interaction, and the pulse height is not proportional to the number of original electrons produced. Therefore, Geiger counters are incapable of particle identification and energy measurement (spectroscopy). Since the process of charge amplification greatly improves the detector’s signal-to-noise ratio, subsequent electronic amplification is usually not required.
Quenching – Dead Time – Geiger Counters
In a Geiger counter, the fill gas of the chamber is an inert gas that is ionized by incident radiation and a quench gas of 5–10% of an organic vapor or a halogen gas to prevent spurious pulsing by quenching the electron avalanches. The Geiger counter should not give spurious pulses and should recover quickly to the passive state, ready for the next radiation event. Argon and helium are the most frequently used fill gases and allow the detection of alpha, beta, and gamma radiation. He-3 and BF3 (Boron Trifluoride) are the most commonly employed for neutron detection.
However, there is a positively charged gas ion left over for each electron collected in the chamber. These gas ions are heavy compared to an electron and move much more slowly. Free electrons are much lighter than positive ions. Thus, they are drawn toward the positive central electrode much faster than the positive ions drawn to the chamber wall. The resulting cloud of positive ions near the electrode leads to distortions in gas multiplication. Eventually, the positive ions move from the positively charged central wire to the negatively charged wall and are neutralized by gaining an electron. These atoms then return to their ground state by emitting photons which in turn produce further ionization and thereby spurious secondary discharges. The electrons produced by this ionization move toward the central wire and are multiplied en route. This charge pulse is unrelated to the radiation to be detected and can set off a series of pulses. The avalanche’s termination is improved by using “quenching” techniques.
The quenching gas molecules have a weaker affinity for electrons than the chamber gas does; therefore, the ionized atoms of the chamber gas readily take electrons from the quenching gas molecules. Thus, the ionized molecules of quenching gas reach the chamber wall instead of the chamber gas. The ionized molecules of the quenching gas are neutralized by gaining an electron, and the energy liberated does not cause further ionization but causes dissociation of the molecule. This type of quenching is known as self-quenching or internal quenching since tubes stop the discharge without external assistance.
External quenching, sometimes called “active quenching” or “electronic quenching, “is also possible for Geiger counters. Electronic quenching uses simplistic high-speed control electronics to rapidly remove and re-apply the high voltage between the electrodes for a fixed time after each discharge peak to increase the maximum count rate and the tube’s lifetime.
Special Reference: U.S. Department of Energy, Instrumentation and Control. DOE Fundamentals Handbook, Volume 2 of 2. June 1992.
Types of Geiger-Mueller Tubes
Geiger counters are mainly used for portable instrumentation due to their sensitivity, simple counting circuit, and ability to detect low-level radiation. Although the major use of Geiger counters is probably in individual particle detection, they are also found in gamma survey meters. They can detect almost all types of radiation, but there are slight differences in the Geiger-Mueller tube. However, the Geiger-Müller tube produces a pulse output of the same magnitude for all detected radiation, so a Geiger counter with an end window tube cannot distinguish between alpha and beta particles.
There are two main types of Geiger tube construction:
- End-Window type. For alpha and beta particles to be detected by Geiger counters, they must be given a thin window. This “end-window” must be thin enough for the alpha and beta particles to penetrate. However, a window of almost any thickness will prevent an alpha particle from entering the chamber. The window is usually made of mica with a density of about 1.5 – 2.0 mg/cm2 to allow low-energy beta particles (e.g., from carbon-14) to enter the detector. The efficiency reduction for alpha is due to the attenuation effect of the end window, though the distance from the surface being checked also has a significant effect. Ideally, a source of alpha radiation should be less than 10mm from the detector due to attenuation in the air.
- Windowless type. Gamma rays have very little trouble penetrating the metal walls of the chamber. Therefore, Geiger counters may be used to detect gamma radiation and X-rays (thin-walled tubes) collectively known as photons, and for this, the windowless tube is used.
- A thick-walled tube is used for gamma radiation detection above energies of about 25 KeV, and this type generally has an overall wall thickness of about 1-2 mm of chrome steel.
- A thin-walled tube is used for low-energy photons (X-rays or gamma rays) and high-energy beta particles. The transition from thin-walled to thick-walled design takes place at the 300–400 keV energy levels. Above these levels, thick-walled designs are used, and beneath these levels, the direct gas ionization effect is predominant.
Sometimes, a “pancake” design of the Geiger-Mueller tube is preferred. This detector is a flat Geiger tube with a thin mica window of a larger area. Flat Geiger tubes like this are known as “pancake” tubes. Such tubes are fitted with a wire screen to protect them. This design provides a larger detecting area and thus higher efficiency to make checking quicker. However, the pressure of the atmosphere against the low pressure of the fill gas limits the window size due to the limited strength of the window membrane.
Detection of Neutrons using Geiger Counter
Since the neutrons are electrically neutral particles, they are mainly subject to strong nuclear forces but not electric ones. Therefore, neutrons are not directly ionizing and usually have to be converted into charged particles before they can be detected. Generally, every type of neutron detector must be equipped with a converter (to convert neutron radiation to common detectable radiation) and one of the conventional radiation detectors (scintillation detector, gaseous detector, semiconductor detector, etc.).
It is not common, but Geiger counters may also be used for neutron detection. In this case, the Geiger-Mueller tube must have the inside of the tube coated with boron, or the tube must contain boron trifluoride (BF3) or helium-3 as the fill gas.