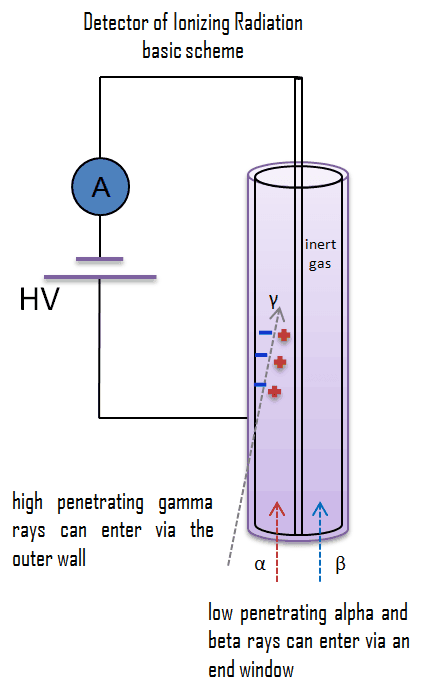
The ionization chamber, also known as the ion chamber, is an electrical device that detects various types of ionizing radiation. The voltage of the detector is adjusted so that the conditions correspond to the ionization region, and the voltage is insufficient to produce gas amplification (secondary ionization).
Advantages of Ionization Chambers
- Current mode. Ionization chambers are preferred for high radiation dose rates because they have no “dead time,” a phenomenon that affects the accuracy of the Geiger-Mueller tube at high dose rates. This is because there is no inherent amplification of signal in the operating medium; therefore, these counters do not require much time to recover from large currents. In addition, because there is no amplification, they provide excellent energy resolution, which is limited primarily by electronic noise. Ionization chambers can be operated in current or pulse mode. In contrast, pulse mode almost always uses proportional counters or Geiger counters. Detectors of ionizing radiation can be used both for activity measurements as well as for dose measurements. The dose can be obtained with knowledge about the energy needed to form a pair of ions. The flat plate design is preferred because it has a well-defined active volume and ensures that ions will not collect on the insulators and cause a distortion of the electric field.
- Simplicity. Output current is independent of detector operating voltage. Observe the flat region of the curve in the ion chamber region. As a result, less regulated and thereby less expensive and more portable power supplies can be used with ion chamber instruments and still offer a reasonably accurate response.
- Neutron Detection. In nuclear reactors, ionization chambers in a current mode often detect neutrons and belong to the Neutron Instrumentation System (NIS). For example, if the inner surface of the ionization chamber is coated with a thin coat of boron, the (n, alpha) reaction can occur. Most of (n,alpha) reactions of thermal neutrons are 10B(n,alpha)7Li reactions accompanied by 0.48 MeV gamma emission. Moreover, isotope boron-10 has a high (n, alpha) reaction cross-section along the entire neutron energy spectrum. The alpha particle causes ionization within the chamber, and ejected electrons cause further secondary ionizations. Another method for detecting neutrons using an ionization chamber is to use the gas boron trifluoride (BF3) instead of air in the chamber. The incoming neutrons produce alpha particles when reacting with the boron atoms in the detector gas. Either method may be used to detect neutrons in a nuclear reactor.
Disadvantages of Ionization Chambers
- No Charge Amplification. Detectors in the ionization region operate at a low electric field strength, so no gas multiplication occurs. The charge collected (output signal) is independent of the applied voltage. Single minimum-ionizing particles tend to be quite small and usually require special low-noise amplifiers for efficient operating performance. The average energy needed to produce an ion in the air is about 34 eV. Therefore a 1 MeV radiation completely absorbed in the detector produces about 3 x 104 pairs of ions. However, it is a small signal that can be considerably amplified using standard electronics. A current of 1 micro-ampere consists of about 1012 electrons per second.
- Low Density. Gamma rays deposit a significantly lower amount of energy into the detector than other particles. Using a high-pressure gas can further increase the chamber’s efficiency.
- For alpha and beta particles to be detected by ionization chambers, they must be given a thin window. This “end-window” must be thin enough for the alpha and beta particles to penetrate. However, a window of almost any thickness will prevent an alpha particle from entering the chamber. The window is usually made of mica with a density of about 1.5 – 2.0 mg/cm2.
Ionization Region
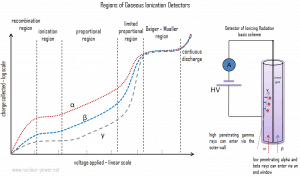
In the ionization region, an increase in voltage does not cause a substantial increase in the number of ion pairs collected. The number of ion pairs collected by the electrodes equals the number of ion pairs produced by the incident radiation. It is dependent on the type and energy of the particles or rays in the incident radiation. Therefore, in this region, the curve is flat. The voltage must be higher than the point where dissociated ion pairs can recombine. On the other hand, the voltage is not high enough to produce gas amplification (secondary ionization). Detectors in the ionization region operate at a low electric field strength, so no gas multiplication occurs, and their current is independent of the applied voltage. They are preferred for high radiation dose rates because they have no “dead time,” a phenomenon that affects the accuracy of the Geiger-Mueller tube at high dose rates.