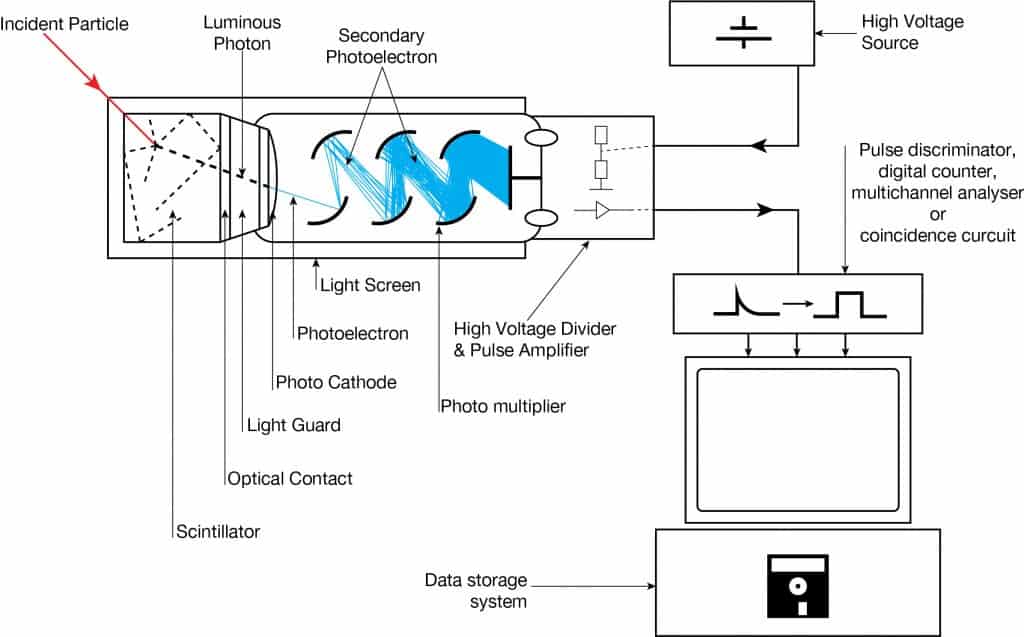
A scintillation counter or scintillation detector is a radiation detector that uses the effect known as scintillation. Scintillation is a flash of light produced in a transparent material by passing a particle (an electron, an alpha particle, an ion, or a high-energy photon). Scintillation occurs in the scintillator, a key part of a scintillation detector. In general, a scintillation detector consists of:
- Scintillator. A scintillator generates photons in response to incident radiation.
- Photodetector. A sensitive photodetector (usually a photomultiplier tube (PMT), a charge-coupled device (CCD) camera, or a photodiode) converts the light to an electrical signal, and electronics process this signal.
The basic principle of operation involves the radiation reacting with a scintillator, which produces a series of flashes of varying intensity. The intensity of the flashes is proportional to the energy of the radiation, and this feature is very important. These counters are suited to measure the energy of gamma radiation (gamma spectroscopy) and, therefore, can be used to identify gamma-emitting isotopes.
Scintillation counters are widely used in radiation protection, an assay of radioactive materials, and physics research because they can be made inexpensively yet with good efficiency and can measure both the intensity and the energy of incident radiation. Hospitals worldwide have gamma cameras based on the scintillation effect; therefore, they are also called scintillation cameras.
The advantages of a scintillation counter are its efficiency and possible high precision and counting rates. These latter attributes result from the extremely short duration of the light flashes, from about 10-9 (organic scintillators) to 10-6 (inorganic scintillators) seconds. The intensity of the flashes and the amplitude of the output voltage pulse are proportional to the energy of the radiation. Therefore, scintillation counters can be used to determine the energy and the number of the exciting particles (or gamma photons). For gamma spectrometry, the most common detectors include sodium iodide (NaI) scintillation counters and high-purity germanium detectors.
Scintillation Counter-Principle of Operation
The operation of scintillation counters is summarized in the following points:
-
Scintillation Counter-Principle of Operation. Source: wikipedia.org License: Public Domain Ionizing radiation enters the scintillator and interacts with the scintillator material, and this causes electrons to be raised to an excited state.
- For charged particles, the track is the path of the particle itself.
- For gamma rays (uncharged), their energy is converted to an energetic electron via either the photoelectric effect, Compton scattering, or pair production.
- The excited atoms of the scintillator material de-excite and rapidly emit a photon in the visible (or near-visible) light range. The quantity is proportional to the energy deposited by the ionizing particle, and the material is said to fluoresce.
- Three classes of phosphors are used:
- inorganic crystals,
- organic crystals,
- plastic phosphors.
- The light created in the scintillator strikes the photocathode of a photomultiplier tube, releasing at most one photoelectron per photon.
- Using a voltage potential, this group of primary electrons is electrostatically accelerated and focused so that they strike the first dynode with enough energy to release additional electrons.
- These secondary electrons are attracted and strike a second dynode releasing more electrons. This process occurs in the photomultiplier tube.
- Each subsequent dynode impact releases further electrons, so there is a current amplifying effect at each dynode stage. Each stage is at a higher potential than the previous to provide the accelerating field.
- The primary signal is multiplied, and this amplification continues through 10 to 12 stages.
- At the final dynode, sufficient electrons are available to produce a pulse of sufficient magnitude for further amplification. This pulse carries information about the energy of the original incident radiation, and the number of such pulses per unit of time also gives information about the intensity of the radiation.
A scintillation detector or scintillation counter is obtained when a scintillator is coupled to an electronic light sensor such as:
- a photomultiplier tube (PMT),
- a charge-coupled device (CCD) camera,
- photodiode
All these devices may be used in scintillation counters, and all convert the light to an electrical signal and contain electronics to process this signal. A photomultiplier tube (PMT) absorbs the light emitted by the scintillator and re-emits it in the form of electrons via the photoelectric effect. The PMT has been the main choice for photon detection ever since because they have high quantum efficiency and high amplification. Lately, however, semiconductors have begun to compete with the PMT. The photodiode, for example, has higher quantum efficiency in the visible range and above, lower power consumption, and smaller size.
Vacuum photodiodes are similar but do not amplify the signal, while silicon photodiodes, on the other hand, detect incoming photons by the excitation of charge carriers directly in the silicon.
Many portable gamma cameras for medical imaging use scintillator-CCD-based detectors. In this case, a scintillator converts the incident radiation (X-rays usually) into visible wavelength photons, which the CCD camera can directly detect.
Note that the term quantum efficiency (QE) may apply to a photosensitive device’s incident photon to converted electron (IPCE) ratio. The quantum efficiency for the photodiode is high (60-80%) compared to the PMT (20-30%), which gives a higher energy resolution.
Scintillation Materials – Scintillators
Scintillators are kinds of materials that provide detectable photons in the visible part of the light spectrum, following the passage of a charged particle or a photon. The scintillator consists of a transparent crystal, usually a phosphor, plastic, or organic liquid, that fluoresces when struck by ionizing radiation. The scintillator must also be transparent to its light emissions and have a short decay time. The scintillator must also be shielded from ambient light so that external photons do not swamp the ionization events caused by incident radiation. A thin opaque foil, such as aluminized mylar, is often used to achieve this. However, it must have a low enough mass to minimize undue attenuation of the measured incident radiation.
There are primarily two types of scintillators commonly used in nuclear and particle physics: organic or plastic scintillators and inorganic or crystalline scintillators.
Inorganic Scintillators
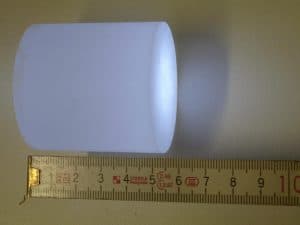
Inorganic scintillators are usually crystals grown in high-temperature furnaces. They include lithium iodide (LiI), sodium iodide (NaI), cesium iodide (CsI), and zinc sulfide (ZnS). NaI(Tl) (thallium-doped sodium iodide) is the most widely used scintillation material. The iodine provides most of the stopping power in sodium iodide (since it has a high Z = 53). These crystalline scintillators are characterized by high density, high atomic number, and pulse decay times of approximately 1 microsecond (~ 10-6 sec). Scintillation in inorganic crystals is typically slower than in organic ones. They exhibit high efficiency for the detection of gamma rays and are capable of handling high count rates. Inorganic crystals can be cut to small sizes and arranged in an array configuration to provide position sensitivity. This feature is widely used in medical imaging to detect X-rays or gamma rays. Inorganic scintillators are better at detecting gamma rays and X-rays than organic scintillators. This is due to their high density and atomic number, which gives a high electron density. A disadvantage of some inorganic crystals, e.g., NaI, is their hygroscopicity, a property that requires them to be housed in an airtight container to protect them from moisture.
Organic Scintillators
Organic scintillators are kinds of organic materials that provide detectable photons in the visible part of the light spectrum, following the passage of a charged particle or a photon. The scintillation mechanism in organic materials is quite different from the mechanism in inorganic crystals. In inorganic scintillators, e.g., NaI, CsI, the scintillation arises because of the structure of the crystal lattice. The fluorescence mechanism in organic materials arises from transitions in the energy levels of a single molecule. Therefore the fluorescence can be observed independently of the physical state (vapor, liquid, solid).
Organic scintillators generally have fast decay times (typically ~10-8 sec), while inorganic crystals are usually far slower (~10-6 sec), although some also have fast components in their response. There are three types of organic scintillators:
- Pure organic crystals. Pure organic crystals include crystals of anthracene, stilbene, and naphthalene. The decay time of this type of phosphor is approximately 10 nanoseconds. This type of crystal is frequently used in the detection of beta particles. They are durable, but their response is anisotropic (which spoils energy resolution when the source is not collimated). They cannot be easily machined, nor can they be grown in large sizes. Therefore they are not very often used.
- Liquid organic solutions. Liquid organic solutions are produced by dissolving an organic scintillator in a solvent.
- Plastic scintillators. Plastic phosphors are made by adding scintillation chemicals to a plastic matrix. The decay constant is the shortest of the three phosphor types, approaching 1 or 2 nanoseconds. Plastic scintillators are more appropriate for use in high-flux environments and high dose rate measurements. The plastic has a high hydrogen content. Therefore, it is useful for fast neutron detectors. It takes substantially more energy to produce a detectable photon in a scintillator than in an electron-ion pair through ionization (typically by a factor of 10). Because inorganic scintillators produce more light than organic scintillators, they are better for applications at low energies.
Photomultiplier Tube
Photomultiplier tubes (PMTs) are photon detection device that uses the photoelectric effect combined with secondary emission to convert light into an electrical signal. A photomultiplier absorbs light emitted by the scintillator and re-emits it in the form of electrons via the photoelectric effect. The PMT has been the main choice for photon detection ever since because they have high quantum efficiency and high amplification.
Components of Photomultiplier Tube
The device consists of several components, and these components are shown in the figure.
-
Apparatus with a scintillating crystal, photomultiplier, and data acquisition components. Source: wikipedia.org License CC BY-SA 3.0 Photocathode. Right after a thin entry window is a photocathode made of material in which the valence electrons are weakly bound and have a high cross-section for converting photons to electrons via the photoelectric effect. For example, Cs3Sb (cesium-antimony) may be used. As a result, the light created in the scintillator strikes the photocathode of a photomultiplier tube, releasing at most one photoelectron per photon.
- Dynodes. Using a voltage potential, this group of primary electrons is electrostatically accelerated and focused so that they strike the first dynode with enough energy to release additional electrons. There is a series (“stages”) of dynodes made of relatively low work function material. These electrodes are operated at increasing potential (e.g., ~100-200 V between dynodes). At the dynode, the electrons are multiplied by secondary emission. The next dynode has a higher voltage which makes the electrons released from the first accelerate towards it. At each dynode, 3-4 electrons are released for every incident electron, and with 6 to 14 dynodes, the total gain, or electron amplification factor, will be in the range of ~104-107 when they reach the anode. Typical operating voltages are in the range of 500 to 3000 V. At the final dynode, and sufficient electrons are available to produce a pulse of sufficient magnitude for further amplification. This pulse carries information about the energy of the original incident radiation, and the number of such pulses per unit of time also gives information about the intensity of the radiation.
Quantum Efficiency
The sensitivity of a photocathode is usually quoted in terms of quantum efficiency. In general, the term quantum efficiency (QE) may apply to a photosensitive device’s incident photon to converted electron (IPCE) ratio. The quantum efficiency of the photocathode is defined as the probability for the conversion of incident photons to an electrical signal and is defined as:
The quantum efficiency of any photosensitive device is a strong function of the wavelength of the incident light, and an effort is made to match the spectral response of the photocathode to the emission spectrum of the scintillator in use. In the photomultiplier tube, the quantum efficiency is limited to 20-30%, but an average quantum efficiency over the emission spectrum of a typical scintillator is about 15-20%.
The standard for quotation is the number of photoelectrons per keV energy loss by fast electrons in a NaI(Tl) scintillator. About 8 ~ 10 photoelectrons are produced per keV energy loss for the peak quantum efficiency. Therefore, the average energy loss required to create a single photoelectron is ~ 100 eV, much bigger than the values in gas-filled or semiconductor detectors.
The PMT has been the main choice for photon detection ever since because they have high quantum efficiency and high amplification. Lately, however, semiconductors have begun to compete with the PMT. The photodiode, for example, has higher quantum efficiency in the visible range and above, lower power consumption, and smaller size. The quantum efficiency for the photodiode is high (60-80%) compared to the PMT (20-30%), which gives a higher energy resolution.
Photodiodes – Scintillation Counter
A scintillation detector or scintillation counter is obtained when a scintillator is coupled to an electronic light sensor such as:
- a photomultiplier tube (PMT),
- a charge-coupled device (CCD) camera,
- photodiode
All these devices may be used in scintillation counters, and all convert the light to an electrical signal and contain electronics to process this signal. A photodiode is a semiconductor device that converts light into an electrical current. This semiconductor device consists of a thin layer of silicon in which the light is absorbed, after which free charge carriers (electrons and holes) are created. A conventional photodiode most often refers to a PIN diode. PIN means that the p and the n doped sides are separated by a depleted i-region. Electrons and holes are collected at the anode and cathode of the diode. This results in a photocurrent that is the output of the diode. The charge is, however, not amplified, making the output signal amplitude small. This makes the photodiode sensitive to electronic noise. On the other hand, the quantum efficiency for the photodiode is high (60-80%) compared to the PMT (20-30%), which gives a higher energy resolution.
Detection of Alpha, Beta, and Gamma Radiation using Scintillation Counter
Scintillation counters are used to measure radiation in various applications, including hand-held radiation survey meters, personnel and environmental monitoring for radioactive contamination, medical imaging, radiometric assay, nuclear security, and nuclear plant safety. They are widely used because they can be made inexpensively yet with good efficiency and can measure both the intensity and the energy of incident radiation.
Scintillation counters can be used to detect alpha, beta, and gamma radiation, and they can also be used for the detection of neutrons. For these purposes, different scintillators are used:
- Alpha Particles and Heavy Ions. Due to the very high ionizing power of heavy ions, scintillation counters are usually not ideal for the detection of heavy ions. For equal energies, a proton will produce 1/4 to 1/2 the light of an electron, while alpha particles will produce only about 1/10 the light. Where needed, inorganic crystals, e.g., CsI(Tl) and ZnS(Ag) (typically used in thin sheets as α-particle monitors), should be preferred to organic materials. Pure CsI is a fast and dense scintillating material with a relatively low light yield that increases significantly with cooling. The drawbacks of CsI are a high-temperature gradient and a slight hygroscopicity.
- Beta Particles. For the detection of beta particles, organic scintillators can be used. Pure organic crystals include crystals of anthracene, stilbene, and naphthalene. The decay time of this type of phosphor is approximately 10 nanoseconds. This type of crystal is frequently used in the detection of beta particles. Organic scintillators, having a lower Z than inorganic crystals, are best suited for detecting low-energy (< 10 MeV) beta particles.
- Gamma Rays. High-Z materials are best suited as scintillators for the detection of gamma rays. NaI(Tl) (thallium-doped sodium iodide) is the most widely used scintillation material. The iodine provides most of the stopping power in sodium iodide (since it has a high Z = 53). These crystalline scintillators are characterized by high density, high atomic number, and pulse decay times of approximately 1 microsecond (~ 10-6 sec). Scintillation in inorganic crystals is typically slower than in organic ones. They exhibit high efficiency for the detection of gamma rays and are capable of handling high count rates. Inorganic crystals can be cut to small sizes and arranged in an array configuration to provide position sensitivity. This feature is widely used in medical imaging to detect X-rays or gamma rays. Inorganic scintillators are better at detecting gamma rays and X-rays. This is due to their high density and atomic number, which gives a high electron density.
- Neutrons. Since the neutrons are electrically neutral particles, they are mainly subject to strong nuclear forces but not electric ones. Therefore, neutrons are not directly ionizing and usually have to be converted into charged particles before they can be detected. Generally, every type of neutron detector must be equipped with a converter (to convert neutron radiation to common detectable radiation) and one of the conventional radiation detectors (scintillation detector, gaseous detector, semiconductor detector, etc.). Fast neutrons (>0.5 MeV) primarily rely on the recoil proton in (n,p) reactions. Materials rich in hydrogen, such as plastic scintillators, are best suited for their detection. Thermal neutrons rely on nuclear reactions such as the (n,γ) or (n,α) reactions to produce ionization. Materials such as LiI(Eu) or glass silicates are therefore particularly well-suited for detecting thermal neutrons.
Gamma Spectroscopy using Scintillation Counter
See also: Gamma Spectroscopy using Scintillation Counter
See also: Gamma Spectroscopy
Gamma spectroscopy generally studies the energy spectra of gamma-ray sources, such as in the nuclear industry, geochemical investigation, and astrophysics. Spectroscopes, or spectrometers, are sophisticated devices designed to measure the spectral power distribution of a source. The incident radiation generates a signal that determines the incident particle’s energy.
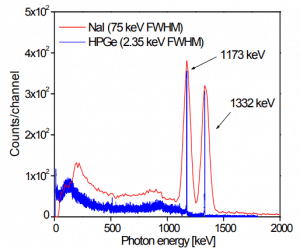
Most radioactive sources produce gamma rays of various energies and intensities. Gamma rays frequently accompany the emission of alpha and beta radiation. Gamma-ray spectrum energy can be produced when these emissions are detected and analyzed with a spectroscopy system. Gamma rays from radioactive decay are in the energy range from a few keV to ~8 MeV, corresponding to the typical energy levels in nuclei with reasonably long lifetimes. As was written, they are produced by the decay of nuclei as they transition from a high energy state to a lower state. A detailed spectrum analysis is typically used to determine the identity and quantity of gamma emitters present in a sample. It is a vital tool in the radiometric assay. The gamma spectrum is characteristic of the gamma-emitting nuclides contained in the source.