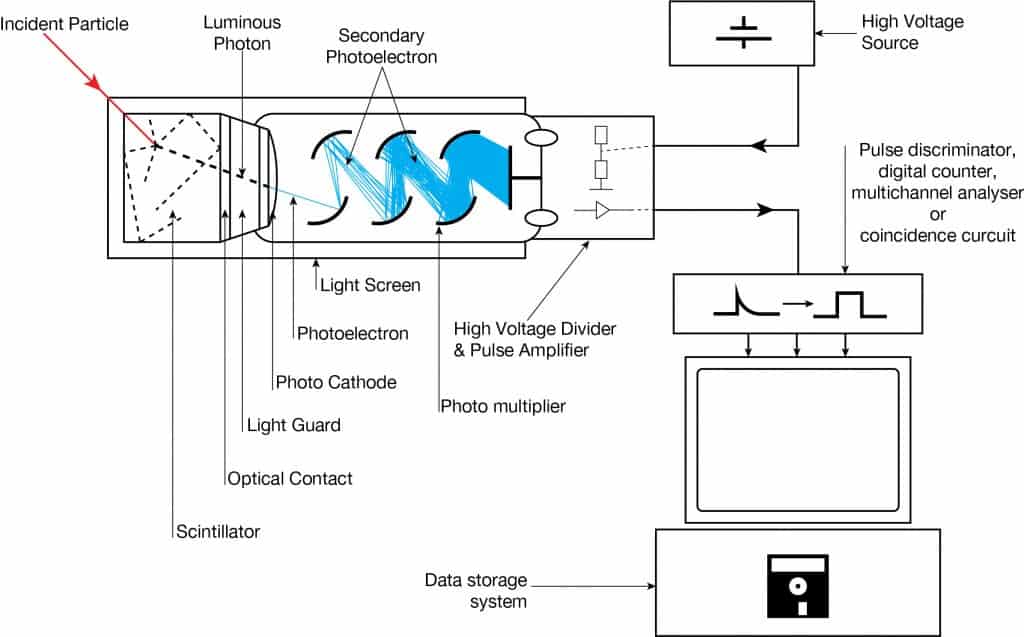
A NaI(Tl) scintillation counter is a radiation detector that uses the effect known as scintillation. Scintillation, which occurs in the NaI(Tl) crystal, is a flash of light produced in a transparent material by the passage of a particle (an electron, an alpha particle, an ion, or a high-energy photon). Scintillation occurs in the scintillator, a key part of a scintillation detector. In general, a scintillation detector consists of:
- Scintillator. A scintillator generates photons in response to incident radiation.
- Photodetector. A sensitive photodetector (usually a photomultiplier tube (PMT), a charge-coupled device (CCD) camera, or a photodiode) converts the light to an electrical signal, and electronics process this signal.
The basic principle of operation involves the radiation reacting with a scintillator, which produces a series of flashes of varying intensity. The intensity of the flashes is proportional to the energy of the radiation, and this feature is very important. These counters are suited to measure the energy of gamma radiation (gamma spectroscopy) and, therefore, can be used to identify gamma-emitting isotopes.
Scintillation counters are widely used in radiation protection, an assay of radioactive materials, and physics research because they can be made inexpensively yet with good efficiency and can measure both the intensity and the energy of incident radiation. Hospitals worldwide have gamma cameras based on the scintillation effect; therefore, they are also called scintillation cameras.
The advantages of a scintillation counter are its efficiency and possible high precision and counting rates. These latter attributes result from the extremely short duration of the light flashes, from about 10-9 (organic scintillators) to 10-6 (inorganic scintillators) seconds. The intensity of the flashes and the amplitude of the output voltage pulse are proportional to the energy of the radiation. Therefore, scintillation counters can be used to determine the energy and the number of the exciting particles (or gamma photons). For gamma spectrometry, the most common detectors include sodium iodide (NaI) scintillation counters and high-purity germanium detectors.
Thallium-doped Sodium Iodide – NaI(Tl) scintillators
NaI(Tl) (thallium-doped sodium iodide) is the most widely used scintillation material. NaI(Tl) as the scintillator is used in scintillation detectors, traditionally in nuclear medicine, geophysics, nuclear physics, and environmental measurements. The iodine provides most of the stopping power in sodium iodide (since it has a high Z = 53). These crystalline scintillators are characterized by high density, high atomic number, and pulse decay times of approximately 1 microsecond (~ 10-6 sec). The wavelength of maximum emission is 415 nm. Scintillation in inorganic crystals is typically slower than in organic ones. They exhibit high efficiency for the detection of gamma rays and are capable of handling high count rates. Inorganic crystals can be cut to small sizes and arranged in an array configuration to provide position sensitivity. This feature is widely used in medical imaging to detect X-rays or gamma rays. Inorganic scintillators are better at detecting gamma rays and X-rays. The NaI(Tl) scintillator has a higher energy resolution than a proportional counter, allowing more accurate energy determinations. This is due to their high density and atomic number, which gives a high electron density. A disadvantage of some inorganic crystals, e.g., NaI, is their hygroscopicity, a property that requires them to be housed in an airtight container to protect them from moisture. The crystals are usually coupled with a photomultiplier tube in a hermetically sealed assembly.
Photomultiplier Tube
Photomultiplier tubes (PMTs) are photon detection device that uses the photoelectric effect combined with secondary emission to convert light into an electrical signal. A photomultiplier absorbs light emitted by the scintillator and re-emits it in the form of electrons via the photoelectric effect. The PMT has been the main choice for photon detection ever since because they have high quantum efficiency and high amplification.
Components of Photomultiplier Tube
The device consists of several components, and these components are shown in the figure.
- Photocathode. Right after a thin entry window is a photocathode made of material in which the valence electrons are weakly bound and have a high cross-section for converting photons to electrons via the photoelectric effect. For example, Cs3Sb (cesium-antimony) may be used. As a result, the light created in the scintillator strikes the photocathode of a photomultiplier tube, releasing at most one photoelectron per photon.
- Dynodes. Using a voltage potential, this group of primary electrons is electrostatically accelerated and focused so that they strike the first dynode with enough energy to release additional electrons. There is a series (“stages”) of dynodes made of relatively low work function material. These electrodes are operated at increasing potential (e.g., ~100-200 V between dynodes). At the dynode, the electrons are multiplied by secondary emission. The next dynode has a higher voltage which makes the electrons released from the first accelerate towards it. At each dynode, 3-4 electrons are released for every incident electron, and with 6 to 14 dynodes, the total gain, or electron amplification factor, will be in the range of ~104-107 when they reach the anode. Typical operating voltages are in the range of 500 to 3000 V. At the final dynode, and sufficient electrons are available to produce a pulse of sufficient magnitude for further amplification. This pulse carries information about the energy of the original incident radiation, and the number of such pulses per unit of time also gives information about the intensity of the radiation.