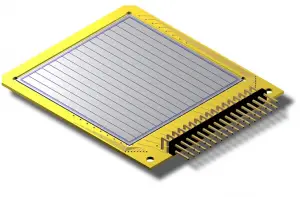
A semiconductor detector is a radiation detector based on a semiconductor, such as silicon or germanium, to measure the effect of incident charged particles or photons. In general, semiconductors are materials, inorganic or organic, which can control their conduction depending on chemical structure, temperature, illumination, and presence of dopants. The name semiconductor comes from the fact that these materials have electrical conductivity between a metal, like copper, gold, etc., and an insulator, such as glass. They have an energy gap of less than 4eV (about 1eV). In solid-state physics, this energy gap or band gap is an energy range between the valence band and conduction band where electron states are forbidden. In contrast to conductors, electrons in a semiconductor must obtain energy (e.g., from ionizing radiation) to cross the band gap and reach the conduction band.
Semiconductor detectors are very similar in operation to photovoltaic panels that generate electric currents. Similarly, a current can be induced by ionizing radiation. As ionizing radiation enters the semiconductor, it interacts with the semiconductor material, and it may excite an electron out of its energy level and consequently leave a hole. This process is known as electron-hole pair generation. In semiconductor detectors, the fundamental information carriers are these electron-hole pairs, which are produced along the path taken by the charged particle (primary or secondary) through the detector. By collecting electron-hole pairs, the detection signal is formed and recorded.
Semiconductor detectors are widely used in radiation protection, an assay of radioactive materials, and physics research because they have some unique features, can be made inexpensively yet with good efficiency, and can measure both the intensity and the energy of incident radiation. These detectors are employed to measure the energy of the radiation and for the identification of particles. If the available semiconductor materials, silicon is mainly used for charged particle detectors (especially for tracking charged particles) and soft X-ray detectors, while germanium is widely used for gamma-ray spectroscopy. A large, clean, and almost perfect semiconductor is ideal as a counter to radioactivity. However, it isn’t easy to make large crystals with sufficient purity. The semiconductor detectors have low efficiency, but they give a very precise measure of energy. Semiconductor detectors, especially germanium-based detectors, are most commonly used where a very good energy resolution is required. The detectors must operate at the very low temperatures of liquid nitrogen (-196°C) to achieve maximum efficiency. Therefore, the drawback is that semiconductor detectors are much more expensive than other detectors and require sophisticated cooling to reduce leakage currents (noise).
Principle of Operation of Semiconductor Detectors
The operation of semiconductor detectors is summarized in the following points:
- Ionizing radiation enters the sensitive volume of the detector and interacts with the semiconductor material.
- Particle passing through the detector ionizes the atoms of the semiconductor, producing the electron-hole pairs. T e number of electron-hole pairs is proportional to the energy of the radiation to the semiconductor. As a result, many electrons are transferred from the valence band to the conduction band, and an equal number of holes are created in the valence band.
- Under the influence of an electric field, electrons and holes travel to the electrodes, where they result in a pulse that can be measured in an outer circuit,
- This pulse carries information about the energy of the original incident radiation. T e number of such pulses per unit time also gives information about the intensity of the radiation.
The energy required to produce electron-hole pairs is very low compared to the energy required to produce paired ions in a gaseous ionization detector. In semiconductor detectors, the statistical variation of the pulse height is smaller, and the energy resolution is higher. As the electrons travel fast, the time resolution is also very good. Compared with gaseous ionization detectors, the density of a semiconductor detector is very high, and charged particles of high energy can give off their energy in a semiconductor of relatively small dimensions.
Silicon-based Semiconductor Detectors
Silicon-based semiconductor detectors are mainly used for charged particle detectors (especially for tracking charged particles) and soft X-ray detectors, while germanium is widely used for gamma-ray spectroscopy. A large, clean, and almost perfect semiconductor is ideal as a counter to radioactivity. However, it isn’t easy to make large crystals with sufficient purity. The semiconductor detectors have low efficiency, but they give a very precise measure of energy. Detectors based on silicon have sufficiently low noise even at room temperature. This is caused by the large band gap of silicon (Egap= 1.12 eV), which allows us to operate the detector at room temperature, but cooling is preferred to reduce noise. T e drawback is that silicon detectors are much more expensive than cloud chambers or wire chambers and require sophisticated cooling to reduce leakage currents (noise). They also suffer degradation over time from radiation. However, this can be greatly reduced thanks to the Lazarus effect.
Application of Silicon Detectors
Since silicon-based detectors are very good for tracking charged particles, they constitute a substantial part of the detection system at the LHC in CERN. M st silicon particle detectors work, in principle, by doping narrow (usually around 100 micrometers wide) strips of silicon to turn them into diodes, which are then reverse biased. A charged particles pass through these strips, and they cause small ionization currents that can be detected and measured. Ranging thousands of these detectors around a collision point in a particle accelerator can yield an accurate picture of what paths particles take. F r example, the Inner Tracking System (ITS) of a Large Ion Collider Experiment (ALICE) contains three layers of silicon-based detectors:
- Silicon Pixel Detector (SPD)
- Silicon Drift Detector (SDD)
- Silicon Strip Detector (SSD)
Silicon Strip Detectors
Silicon-based detectors are very good for tracking charged particles. A silicon strip detector is an arrangement of the strip-like shaped implants acting as charge-collecting electrodes.
Silicon strip detectors 5 x 5 cm2 in the area are quite common and are used in series (just like planes of MWPCs) to determine charged-particle trajectories to position accuracies of the order of several μm in the transverse direction. These implants form a one-dimensional array of diodes on a low-doped, fully depleted silicon wafer. A position-sensitive detector is built by connecting each metalized strip to a charge-sensitive amplifier. Two-dimensional position measurements can be achieved by applying additional strip-like doping on the wafer backside using double-sided technology. Such devices can measure small impact parameters and thereby determine whether some charged particle originated from a primary collision or was the decay product of a primary particle that traveled a small distance from the original interaction and then decayed.
Silicon strip detectors constitute a substantial part of the detection system at the LHC in CERN. Most silicon particle detectors work, in principle, by doping narrow (usually around 100 micrometers wide) strips of silicon to turn them into diodes, which are then reverse biased. As charged particles pass through these strips, they cause small ionization currents that can be detected and measured. Arranging thousands of these detectors around a collision point in a particle accelerator can yield an accurate picture of what paths particles take.
For example, the Inner Tracking System (ITS) of a Large Ion Collider Experiment (ALICE) contains three layers of silicon-based detectors:
- Silicon Pixel Detector (SPD)
- Silicon Drift Detector (SDD)
- Silicon Strip Detector (SSD)
Delta E – E Detector – Telescope
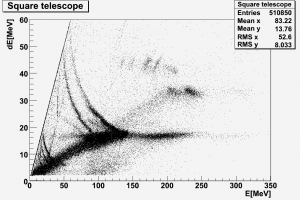
In experimental physics, ΔE-E detectors, known as telescopes, are powerful devices for charged particle identification. Telescopes consisting of pairs of thin and thick surface-barrier detectors can be used to provide charged-particle identification. The e detectors must be positioned in series. The velocity is deduced from the stopping power measured in the thin detectors (ΔE detectors). The e is a strong correlation between the energy deposited in each detector. This correlation depends on the mass (A), the charge (Z), and the kinetic energy (E) of each particle. The mass is deduced from the range or the total kinetic energy loss in the thicker detector (E detector).
Telescopes can be composed of several detectors (ionization chambers, silicon detectors, and scintillators, for instance) stacked to slow down incident-charged particles, the first detector being the thinnest and the last one the thickest. CsI scintillation counters can be, for example, used as final E counters. As an example of the telescope, an assembly based on two front ΔE silicon detectors (10 or 30 µm) and an E silicon counter 1500 µm thick may be used to detect high-energy charged particles.
Germanium-based Semiconductor Detectors
Germanium-based semiconductor detectors are most commonly used where a very good energy resolution is required, especially for gamma spectroscopy as well as x-ray spectroscopy. In gamma spectroscopy, germanium is preferred due to its atomic number being much higher than silicon, increasing the probability of gamma-ray interaction. Mor over, germanium has lower average energy necessary to create an electron-hole pair, which is 3.6 eV for silicon and 2.9 eV for germanium. This also provides the latter with a better resolution in energy. A large, clean, and almost perfect germanium semiconductor is ideal as a counter to radioactivity. However, making large crystals with sufficient purity is difficult and expensive. Whi e silicon-based detectors cannot be thicker than a few millimeters, and germanium can have a depleted, sensitive thickness of centimeters and, therefore, can be used as a total absorption detector for gamma rays up to a few MeV.
On the other hand, to achieve maximum efficiency, the detectors must operate at the very low temperatures of liquid nitrogen (-196°C) because, at room temperatures, the noise caused by thermal excitation is very high.
Since germanium detectors produce the highest resolution commonly available today, they are used to measure radiation in various applications, including personnel and environmental monitoring for radioactive contamination, medical applications, radiometric assay, nuclear security, and nuclear plant safety.
Application of Germanium Detectors – Gamma Spectroscopy
As was written, gamma spectroscopy is used to study and analyze gamma-ray spectra for scientific and technical use. Gamma-ray spectrometers are the instruments that observe and collect such data. A gamma-ray spectrometer (GRS) is a sophisticated device for measuring the energy distribution of gamma radiation. For the measurement of gamma rays above several hundred keV, there are two detector categories of major importance, inorganic scintillators such as NaI(Tl) and semiconductor detectors. In the previous articles, we described gamma spectroscopy using a scintillation detector consisting of a suitable scintillator crystal, a photomultiplier tube, and a circuit for measuring the height of the pulses produced by the photomultiplier. The advantages of a scintillation counter are its efficiency (large size and high density) and the possible high precision and counting rates. Due to the high atomic number of iodine, a large number of interactions will result in the complete absorption of gamma-ray energy so that the photo fraction will be high.
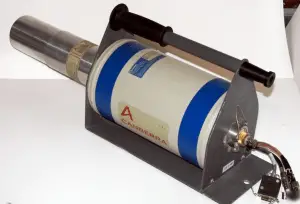
But if a perfect energy resolution is required, we must use a germanium-based detector, such as the HPGe detector. Germanium-based semiconductor detectors are most commonly used where a very good energy resolution is required, especially for gamma and x-ray spectroscopy. In gamma spectroscopy, germanium is preferred due to its atomic number being much higher than silicon, increasing the probability of gamma-ray interaction. Mor over, germanium has lower average energy necessary to create an electron-hole pair, which is 3.6 eV for silicon and 2.9 eV for germanium. This also provides the latter with a better resolution in energy. The FWHM (full width at half maximum) for germanium detectors is an energy function. For a 1.3 MeV photon, the FWHM is 2.1 keV, which is very low.