X-rays, also known as X-radiation, refer to electromagnetic radiation (no rest mass, no charge) of high energies. X-rays are high-energy photons with short wavelengths and thus very high frequency. The radiation frequency is the key parameter of all photons because it determines the energy of a photon. Photons are categorized according to their energies, from low-energy radio waves and infrared radiation, through visible light, to high-energy X-rays and gamma rays.
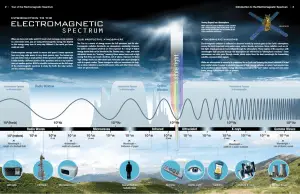
Most X-rays have a wavelength ranging from 0.01 to 10 nanometers (3×1016 Hz to 3×1019 Hz), corresponding to energies from 100 eV to 100 keV. X-ray wavelengths are shorter than those of UV rays and typically longer than those of gamma rays. The distinction between X-rays and gamma rays is not so simple and has changed in recent decades. According to the currently valid definition, X-rays are emitted by electrons outside the nucleus, while gamma rays are emitted by the nucleus.
Since the X-rays (especially hard X-rays) are in substance high-energy photons, they are very penetrating matter and are thus biologically hazardous. X-rays can travel thousands of feet in the air and easily pass through the human body.
Discovery of X-Rays – Wilhelm Conrad Röntgen
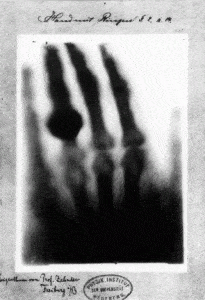
Source: wikipedia.org License: Public Domain
X-rays were discovered on November 8, 1895, by German physics professor Wilhelm Conrad Röntgen at the University of Würtzburg in Germany. He studied electric discharges in glass tubes filled with various gases at very low pressures. In these experiments, Röntgen had covered the tube with some black paper and hand darkened the room. He then discovered that a piece of paper painted with a fluorescent dye would glow when he turned on the high voltage between the electrodes in the tube at some distance from the tube. He realized that he had produced a previously unknown “invisible light,” or ray, that was being emitted from the tube and a ray capable of passing through the heavy paper covering the tube. Röntgen referred to the radiation as “X” to indicate that it was an unknown type of radiation.
Realizing the importance of his discovery, Röntgen focused all his attention on studying this new radiation that had the unusual property of passing through black paper. Through additional experiments, he also found that the new ray would pass through most substances casting shadows on solid objects such as blocks of wood, books, and even his hand. He found that X-rays propagate in straight lines from which they are deflected neither by electric nor magnetic fields. The first x-ray image was a picture of his wife’s hand on a photographic plate formed due to X-rays. His discovery spread quickly throughout the world, and Wilhelm Conrad Röntgen received the first Nobel Prize in Physics for his discovery.
Characteristics of X-rays
Key features of X-rays are summarized in the following few points:
- X-rays are high-energy photons (about 100 – 1 000 times as much energy as the visible photons), the same photons as the photons forming the visible range of the electromagnetic spectrum – light.
- X-rays are usually described by their maximum energy, determined by the voltage between the electrodes, and they may range from about 20 kV up to 300 kV. Radiation with low voltage is called “soft,” – and radiation with high voltage is called “hard.”
- Photons (gamma rays and X-rays) can ionize atoms directly (despite they are electrically neutral) through the Photoelectric effect and the Compton effect, but secondary (indirect) ionization is much more significant.
- X-rays ionize matter via indirect ionization.
- Although many possible interactions are known, there are three key interaction mechanisms with the matter.
- Photoelectric effect
- Compton scattering
- Rayleigh scattering
- X-rays travel at the speed of light and can travel hundreds of meters in the air before spending their energy.
- Since the hard X-rays are very penetrating matter, they must be shielded by very dense materials, such as lead or uranium.
- The distinction between X-rays and gamma rays is not so simple and has changed in recent decades. According to the currently valid definition, X-rays are emitted by electrons outside the nucleus, while gamma rays are emitted by the nucleus.
- For X-rays generated by X-ray tube, there are two different types of X-rays spectra:
- Bremsstrahlung
- Characteristic X-rays
- Characteristic X-rays frequently accompany some types of nuclear decays, such as internal conversion and electron capture.
X-ray – Production
Since X-rays are high-energy photons, which have electromagnetic nature, they can be produced whenever charged particles (electrons or ions) of sufficient energy hit a material. It is similar to the photoelectric effect, where photons can be annihilated when they strike the metal plate, each surrendering its kinetic energy to an electron.
X-rays can be generated by an X-ray tube, a vacuum tube that uses a high voltage to accelerate the electrons released by a hot cathode to a high velocity. The cathode must be heated to emit electrons. Electrons, accelerated by potential differences of tens of thousands of volts, are aimed at a metal target (usually made of tungsten or another heavy metal) in a vacuum tube. The larger the voltage between the electrodes, the higher energy will the electrons attain. Upon striking the target, the accelerated electrons are abruptly stopped, and X-rays and heat are generated. Most energy is transformed into heat in the anode (which must be cooled). Just 1% of the kinetic energy of the electrons is converted into X-rays. X-rays are usually generated perpendicular to the path of the electron beam.
A specialized source of X-rays becoming widely used in research is a particle accelerator, which generates radiation known as synchrotron radiation. When ultra-relativistic charged particles move through magnetic fields, they are forced to move along a curved path. Since their direction of motion is continually changing, they are also accelerating and emitting bremsstrahlung. In this case, it is referred to as synchrotron radiation.
X-rays can also be produced by fast protons or other positive ions. The proton-induced X-ray emission or particle-induced X-ray emission is widely used as an analytical procedure.
Soft and Hard X-rays
X-rays are usually described by their maximum energy, determined by the voltage between the electrodes. X-rays with high photon energies (above 5–10 keV) are called hard X-rays, while those with lower energy (and longer wavelength) are called soft X-rays. Due to their penetrating ability, hard X-rays are widely used to image the inside of visually opaque objects. The most often seen applications are in medical radiography. Since the wavelengths of hard X-rays are similar to the size of atoms, they are also useful for determining crystal structures by X-ray crystallography. By contrast, soft X-rays are easily absorbed in the air. The attenuation length of 600 eV X-rays in water is less than 1 micrometer.
X-Ray Spectrum – Characteristic and Continuous
For X-rays generated by an X-ray tube, the part of the energy that is transformed into radiation varies from zero up to the maximum energy of the electron when it hits the anode. The maximum energy of the produced X-ray photon is limited by the energy of the incident electron, which is equal to the voltage on the tube times the electron charge, so a 100 kV tube cannot create X-rays with an energy greater than 100 keV. When the electrons hit the target, X-rays are created by two different atomic processes:
- Bremsstrahlung. The bremsstrahlung is electromagnetic radiation produced by the acceleration or deceleration of an electron when deflected by strong electromagnetic fields of target high-Z (proton number) nuclei. The name bremsstrahlung comes from German, and the literal translation is ‘braking radiation.’ From classical theory, when a charged particle is accelerated or decelerated, it must radiate energy. The bremsstrahlung is one of the possible interactions of light-charged particles with matter (especially with high atomic numbers). These X-rays have a continuous spectrum. The intensity of the X-rays increases linearly with decreasing frequency, from zero at the energy of the incident electrons to the voltage on the X-ray tube. Changing the material from which the target in the tube is made does not affect the spectrum of this continuous radiation. If we were to switch from a molybdenum target to a copper target, all features of the x-ray spectrum would change except the cutoff wavelength.
- Characteristic X-ray emission. If the electron has enough energy, it can knock an orbital electron out of the inner electron shell of a metal atom. Since the process leaves a vacancy in the electron energy level from which the electron came, the outer electrons of the atom cascade down to fill the lower atomic levels, and one or more characteristic X-rays are usually emitted. As a result, sharp intensity peaks appear in the spectrum at wavelengths characteristic of the material from which the anode target is made. The frequencies of the characteristic X-rays can be predicted from the Bohr model.
Interaction of X-rays with Matter
Although many possible interactions are known, there are three key interaction mechanisms with the matter. The strength of these interactions depends on the energy of the X-rays and the elemental composition of the material. Still, not much on chemical properties, since the X-ray photon energy is much higher than chemical binding energies. The photoelectric absorption dominates at low-energies of X-rays, while Compton scattering dominates at higher energies.
- Photoelectric absorption
- Compton scattering
- Rayleigh scattering
Photoelectric Absorption of X-rays
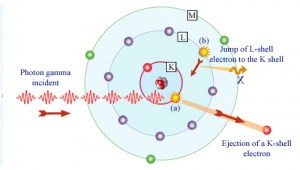
Source: laradioactivite.com/
In the photoelectric effect, a photon undergoes an interaction with an electron that is bound in an atom. In this interaction, the incident photon completely disappears, and an energetic photoelectron is ejected by the atom from one of its bound shells. The kinetic energy of the ejected photoelectron (Ee) is equal to the incident photon energy (hν) minus the binding energy of the photoelectron in its original shell (Eb).
Ee=hν-Eb
Therefore photoelectrons are only emitted by the photoelectric effect if a photon reaches or exceeds threshold energy – the binding energy of the electron – the work function of the material. For very high X-rays with energies of more than hundreds of keV, the photoelectron carries off the majority of the incident photon energy – hν.
At small values of gamma-ray energy, the photoelectric effect dominates. The mechanism is also enhanced for materials of high atomic number Z. It is not simple to derive an analytic expression for the probability of photoelectric absorption of gamma-ray per atom over all ranges of gamma-ray energies. The probability of photoelectric absorption per unit mass is approximately proportional to:
τ(photoelectric) = constant x ZN/E3.5
where Z is the atomic number, and the exponent n varies between 4 and 5. E is the energy of the incident photon. The proportionality to higher powers of the atomic number Z is the main reason for using high Z materials, such as lead or depleted uranium in gamma-ray shields.
Although the probability of the photoelectric absorption of a photon decreases, in general, with increasing photon energy, there are sharp discontinuities in the cross-section curve. These are called “absorption edges,” and they correspond to the binding energies of electrons from an atom’s bound shells. For photons with the energy just above the edge, the photon energy is sufficient to undergo the photoelectric interaction with the electron from a bound shell, let’s say K-shell. The probability of such interaction is just above this edge, much greater than that of photons of energy slightly below this edge. For photons below this edge, the interaction with an electron from K-shell is energetically impossible, and therefore the probability drops abruptly. These edges also occur at binding energies of electrons from other shells (L, M, N …).
Compton Scattering of X-rays
The Compton formula was published in 1923 in the Physical Review. Compton explained that the X-ray shift is caused by the particle-like momentum of photons. Compton scattering formula is the mathematical relationship between the shift in wavelength and the scattering angle of the X-rays. In the case of Compton scattering, the photon of frequency f collides with an electron at rest. Upon collision, the photon bounces off the electron, giving up some initial energy (given by Planck’s formula E=hf). While the electron gains momentum (mass x velocity), the photon cannot lower its velocity. As a result of momentum conservation law, the photon must lower its momentum given by:
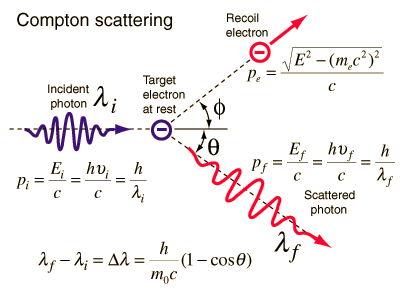
Source: hyperphysics.phy-astr.gsu.edu
So the decrease in photon’s momentum must be translated into a decrease in frequency (increase in wavelength Δλ = λ’ – λ). The shift of the wavelength increased with scattering angle according to the Compton formula:
where λ is the initial wavelength of the photon, λ’ is the wavelength after scattering, h is the Planck constant = 6.626 x 10-34 J.s, and me is the electron rest mass (0.511 MeV), c is the speed of light, Θ is the scattering angle. The minimum change in wavelength (λ′ − λ) for the photon occurs when Θ = 0° (cos(Θ)=1) and is at least zero. The maximum change in wavelength (λ′ − λ) for the photon occurs when Θ = 180° (cos(Θ)=-1). In this case, the photon transfers to the electron as much momentum as possible. The maximum change in wavelength can be derived from the Compton formula:
The quantity h/mec is known as the Compton wavelength of the electron and is equal to 2.43×10−12 m.
Rayleigh Scattering – Thomson Scattering
Rayleigh scattering, also known as Thomson scattering, is the low-energy limit of Compton scattering. The particle kinetic energy and photon frequency do not change due to the scattering. Rayleigh scattering occurs due to an interaction between an incoming photon and an electron, the binding energy of which is significantly greater than that of the incoming photon. The incident radiation is assumed to set the electron into forced resonant oscillation such that the electron re-emits radiation of the same frequency but in all directions. In this case, the electric field of the incident wave (photon) accelerates the charged particle, causing it, in turn, to emit radiation at the same frequency as the incident wave, and thus the wave is scattered. Rayleigh scattering is significant up to ≈ 20keV and elastic like Thomson scattering. The total scattering cross-section combines the Rayleigh and Compton bound scattering cross-sections. Thomson scattering is an important phenomenon in plasma physics and was first explained by the physicist J. J. Thomson. This interaction has great significance in the area of X-ray crystallography.
X-ray Attenuation
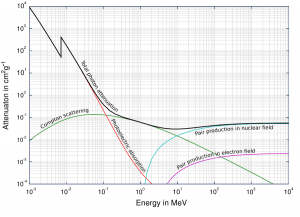
Source: Wikimedia Commons
As the high-energy photons pass through the material, their energy decreases, which is known as attenuation. The attenuation theory is valid for X-rays and gamma rays as well. It turns out that higher energy photons (hard X-rays) travel through tissue more easily than low-energy photons (i.e., the higher energy photons are less likely to interact with matter). Much of this effect is related to the photoelectric effect. The probability of photoelectric absorption is approximately proportional to (Z/E)3, where Z is the atomic number of the tissue atom and E is the photon energy. As E gets larger, the likelihood of interaction drops rapidly. For higher energies, Compton scattering becomes dominant. Compton scattering is about constant for different energies, although it slowly decreases at higher energies.
See also: X-ray Attenuation
Shielding of X-rays
In short, effective shielding of X-rays is, in most cases, based on the use of materials with two following material properties:
- high-density of material.
- the high atomic number of material (high Z materials)
However, low-density materials and low Z materials can be compensated with increased thickness, which is as significant as density and atomic number in shielding applications.
A lead is widely used as an X-ray shield. The major advantage of a lead shield is its compactness due to its higher density. A lead is widely used as a gamma shield. On the other hand, depleted uranium is much more effective due to its higher Z. Depleted uranium is used for shielding in portable gamma-ray sources.
In nuclear power plants, shielding of a reactor core can be provided by materials of reactor pressure vessels and reactor internals (neutron reflector). Also, heavy concrete is usually used to shield neutrons and gamma radiation.
X-ray shielding is more complex and difficult than alpha or beta radiation shielding. To understand comprehensively the way how an X-ray loses its initial energy, how it can be attenuated, and how it can be shielded, we must have detailed knowledge of its interaction mechanisms.
See also more theory: Interaction of X-rays with Matter
See also calculator: Gamma activity to dose rate (with/without shield)
See also XCOM – photon cross-section DB: XCOM: Photon Cross Sections Database