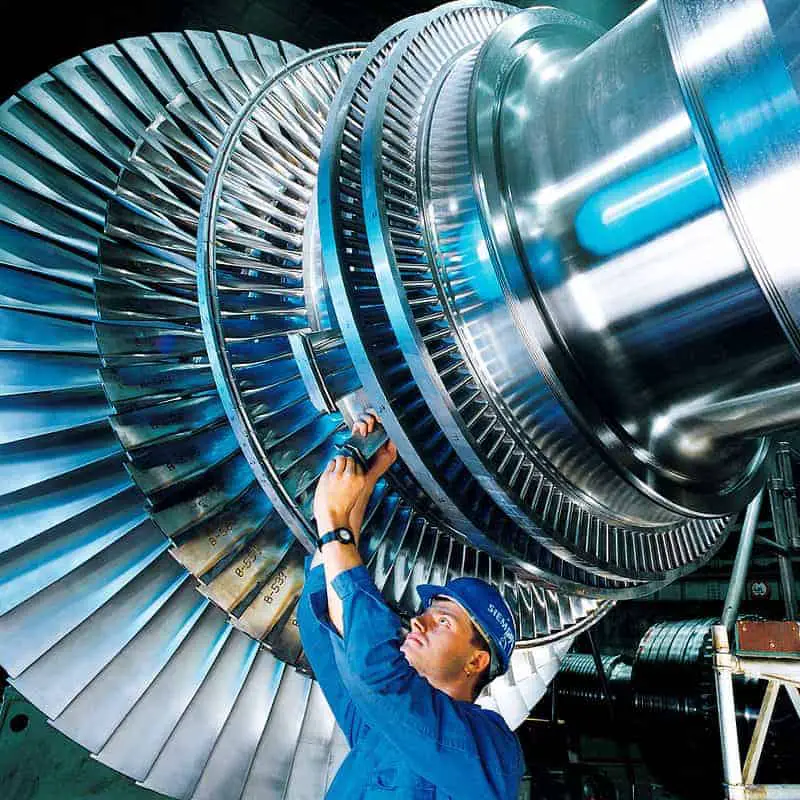
Most nuclear power plants operate a single-shaft turbine generator consisting of one multi-stage HP turbine, three parallel multi-stage LP turbines, the main generator, and an exciter. HP Turbine is usually a double-flow reaction turbine with about 10 stages with shrouded blades and produces about 30-40% of the gross power output of the power plant unit. LP turbines are usually double-flow reaction turbines with about 5-8 stages (with shrouded blades and free-standing blades for the last 3 stages). LP turbines produce approximately 60-70% of the gross power output of the power plant unit. Each turbine rotor is mounted on two bearings, i.e., double bearings between each turbine module. The range of alloys used in steam turbines is relatively small, partly because of the need to ensure a good match of thermal properties, such as expansion and conductivity, and partly because of the need for high-temperature strength at an acceptable cost.
- Material for turbine rotors. The rotors of steam turbines are usually made of low-alloy steel. The role of the alloying elements is to increase hardenability to optimize mechanical properties and toughness after heat treatment. The rotors are required to handle the highest steam conditions; therefore, the alloy most commonly used is CrMoV steel.
- Material for the casing. The casings of steam turbines typically are large structures with complex shapes that must provide the pressure containment for the steam turbine. Because of the size of these components, their cost has a strong impact on the overall cost of the turbine. The materials used currently for inner and outer casings are usually low-alloy CrMo steels (e.g., the 1-2CrMo steel). For higher temperatures, cast 9CrMoVNb alloys are considered adequate in terms of strength.
- Material of turbine blading. For gas turbines, the turbine blades are often the limiting component. The highest temperature in the cycle occurs at the end of the combustion process, and it is limited by the maximum temperature that the turbine blades can withstand. As usual, metallurgical considerations (about 1700 K) place upper limits on thermal efficiency. Therefore turbine blades often use exotic materials like superalloys and many different cooling methods, such as internal air channels, boundary layer cooling, and thermal barrier coatings. The development of superalloys in the 1940s and new processing methods, such as vacuum induction melting in the 1950s, greatly increased the temperature capability of turbine blades. Modern turbine blades often use nickel-based superalloys that incorporate chromium, cobalt, and rhenium.
- Steam turbine blades are not exposed to such high temperatures, but they must withstand an operation with two-phase fluid. The high content of water droplets can cause rapid impingement and erosion of the blades, which occurs when condensed water is blasted onto the blades. For example, condensate drains are installed in the steam piping leading to the turbine to prevent this. Another challenge for engineers is the design of blades for the last stage of the LP turbine. These blades must be (due to the high specific volume of steam) very long, which induces enormous centrifugal forces during operation. Therefore, turbine blades are subjected to stress from centrifugal force (turbine stages can rotate at tens of thousands of revolutions per minute (RPM), but usually at 1800 RPM) and fluid forces that can cause a fracture, yielding, or creep failures.
Material Problems of Turbines
Creep
Creep, also known as cold flow, is the permanent deformation that increases with time under constant load or stress. It results from long-time exposure to large external mechanical stress within the limit of yielding and is more severe in materials subjected to heat for a long time. The rate of deformation is a function of the material’s properties, exposure time, exposure temperature, and the applied structural load. Creep is very important if we use materials at high temperatures. Creep is very important in the power industry and is of the highest importance in designing jet engines. Time to rupture is the dominant design consideration for many relatively short-life creep situations (e.g., turbine blades in military aircraft). Of course, for its determination, creep tests must be conducted to the point of failure, termed creep rupture tests.
Erosion Corrosion
Erosion corrosion is the cumulative damage induced by electrochemical corrosion reactions and mechanical effects from relative motion between the electrolyte and the corroding surface. Erosion can also occur with other forms of degradation, such as corrosion, which is referred to as erosion-corrosion. Erosion corrosion is a material degradation process due to the combined effect of corrosion and wear. Nearly all flowing or turbulent corrosive media can cause erosion corrosion. The mechanism can be described as follows:
- mechanical erosion of the material, or protective (or passive) oxide layer on its surface,
- enhanced corrosion of the material if the corrosion rate of the material depends on the thickness of the oxide layer.
Erosion corrosion is found in the systems such as piping, valves, pumps, nozzles, heat exchangers, and turbines. Wear is a mechanical material degradation process occurring on rubbing or impacting surfaces, while corrosion involves chemical or electrochemical reactions of the material. Corrosion may accelerate wear, and wear may accelerate corrosion.
Steam Oxidation
Steam oxidation behavior is directly linked to implementing ultra-supercritical steam power generation for improved efficiencies and reduced CO2 emissions. Higher temperature means higher efficiency; however, higher corrosion rates occur in a steam atmosphere when ferritic, ferritic‐martensitic, or medium Cr–Ni steels are used.
The materials developed over 50–60 years ago are no longer suitable for ultra-supercritical regimes due to poor corrosion resistance and inadequate high‐temperature creep and strength properties. These technologies require advanced austenitic steels and nickel (Ni)‐based alloys with superior steam oxidation resistance.
Fatigue
In materials science, fatigue is the weakening of a material caused by cyclic loading, resulting in progressive, brittle, and localized structural damage. Once a crack has been initiated, each loading cycle will grow the crack a small amount, even when repeated alternating or cyclic stresses are of an intensity considerably below the normal strength. The stresses could be due to vibration or thermal cycling. Fatigue damage is caused by:
- simultaneous action of cyclic stress,
- tensile stress (whether directly applied or residual),
- plastic strain.
A fatigue crack will not initiate and propagate if any of these three is not present. The majority of engineering failures are caused by fatigue.
Although the fracture is of a brittle type, it may take some time to propagate, depending on the intensity and frequency of the stress cycles. Nevertheless, there is very little warning before failure if the crack is not noticed. The number of cycles required to cause fatigue failure at a particular peak of stress is generally quite large, but it decreases as the stress increases. For some mild steels, cyclical stresses can be continued indefinitely provided the peak stress (sometimes called fatigue strength) is below the endurance limit value. The type of fatigue of most concern in nuclear power plants is thermal fatigue. Thermal fatigue can arise from thermal stresses produced by cyclic changes in temperature. Large components like the pressurizer, reactor vessel, and reactor system piping are subject to cyclic stresses caused by temperature variations during reactor startup, changes in power level, and shutdown.